How Nuclear Reactors Became Big…
- Author : Alain Vallée
- Jul 3, 2020
- 7 min read
Updated: Nov 18, 2020
Once upon a time, all nuclear reactors were small; then, the natural selection process began, and today they are big.
But there has always been a dream for the lost paradise of small reactors, leading to plenty of attempts at breaking the race for power; as of today, SMRs make media news headlines, announcing numerous new initiatives; last year, the IAEA recorded 55 projects at various stages of development. All these projects are searching for a fine blend of technical solutions that can break the race for power.
NucAdvisor, a nuclear consulting company, is quite active in reactor developments, including SMRs, and has accurate knowledge of the challenges faced by a developer in carrying a new project from birth to successful completion. For doing that, it is necessary to have a good understanding of the main drivers that have shaped the nuclear energy history. We shall publish several letters on SMRs, and this is the first one which presents the historical selection process that led to reactors with power significantly above 1 000 MWe, some of them above 1700 MWe.
1950-1974: The Glorious Period of Nuclear Energy
Nuclear electricity generation started in 1954, at Obninsk (former USSR) with a 5 MWe water-graphite reactor (LWGR). It was followed by Calder Hall, in the UK, the first in a series of eight 50-MWe reactors, using gas-cooled, graphite moderated technology (GCR). In 1957, US-AEC put in operation the first 60-MWe pressurized water reactor (PWR) at Shippingport, derived from the submarine nuclear reactor design. It was closely followed (in the same year), also in the US, by the first boiling water reactor (BWR, 24-MWe) at Vallecitos. Then came France, in 1962, with G2, a 39-MWe gas-graphite reactor at Marcoule, and Canada, in 1963, with Rolphton a 21-MWe light water – heavy water reactor (PHWR).
Several other technologies were tested, like high temperature gas cooled reactors (HTGR) or fast-breeder reactors (FBR), but with limited success.
The number of reactors under construction started increasing steadily and, among the technologies, GCR took the lead at the very beginning, drawn by the English and the French programs, until it was overtaken by the rapid deployment of light water reactors (LWR) in the US, which took place during the second half of the sixties (see Figure 1).
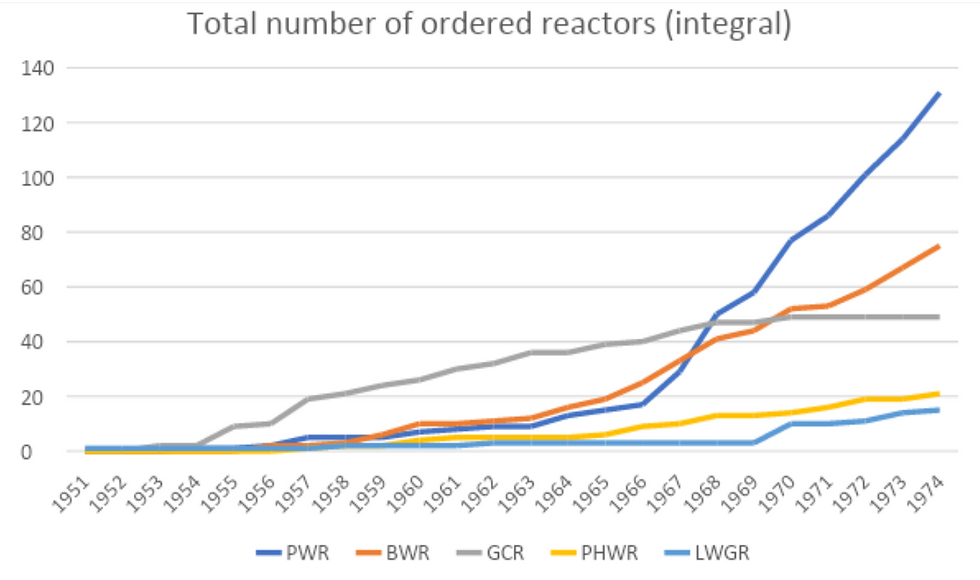
Figure 1: Data IAEA - PRIS
The success of LWRs was due to the conjunction of several factors; a major one was obviously the capabilities of the two major vendors, Westinghouse for PWR, and General Electric for BWR. Both companies had large financial assets and experience of electricity generation with other types of plants, so they were able to design industrial products meeting the needs of the utilities.
During this early phase of development, the driving force was mastering the novel energy sources, and public money was there to invest in such a promising energy. Nevertheless, in the USA, competitiveness was a driver, and, in the second phase, economy became a major factor in technology selection. It appeared quickly that increasing the power of reactors was beneficial for the capital cost (per kW), the operating costs (size of staff, maintenance) and the fuel costs (neutrons leaking out of the core). So, very quickly, the power level started to grow, as shown in Figure 2.
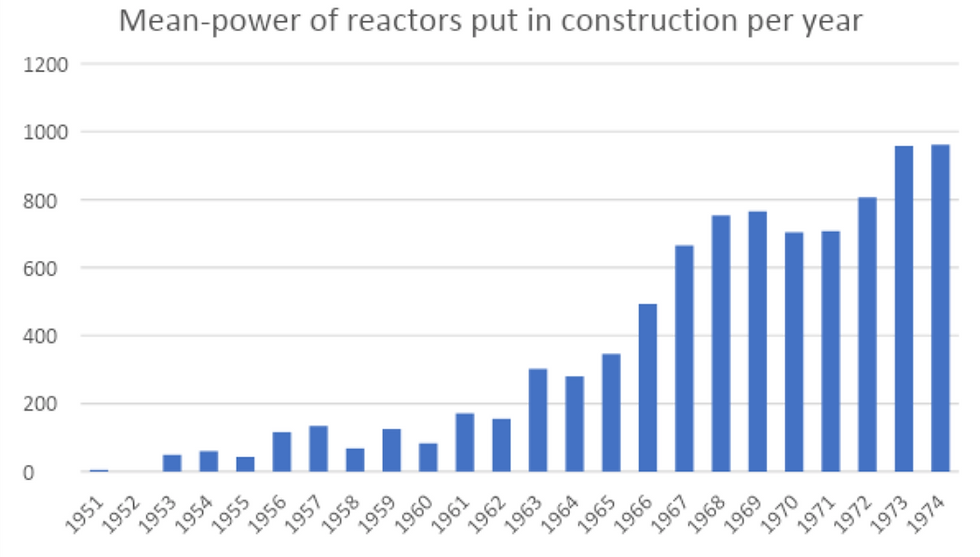
Figure 2: Data IAEA - PRIS
But in the race for power, all technologies were not equal, as shown in Figure 3, below. The limiting factor is the stability of the core, which is linked to its size. The first to reach their limit were the GCRs, which grew up to 600 MWe. The PHWR reached 800 MWe and the Russian LWGR was close to 1,000 MWe, while LWGR was reactors rapidly exceeded 1,200 MWe.
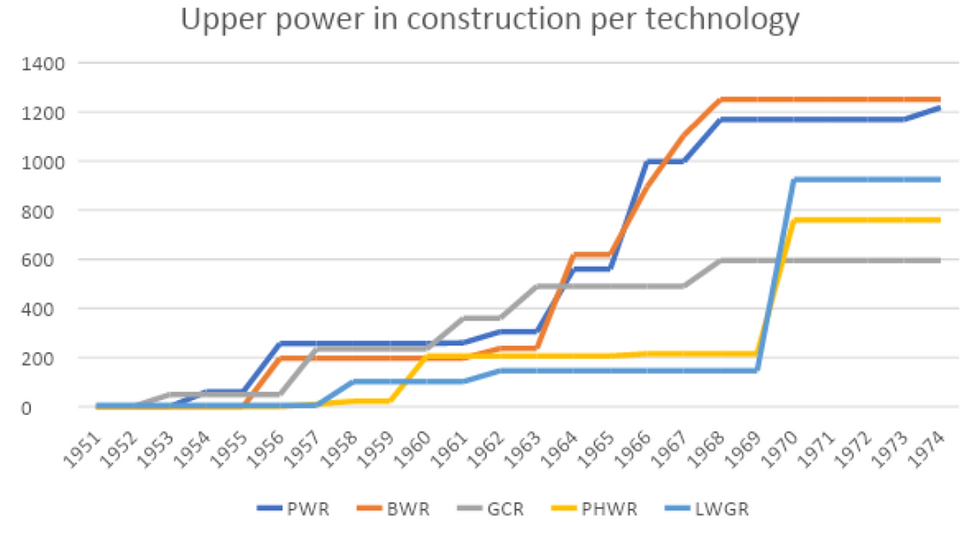
Figure 3: Data IAEA - PRIS
In 1971, France decided to abandon its GCR national program and to switch to the American LWR technology for economic reasons, after detailed cost estimations of the various technologies. The UK, making use of its newly found gas resources, progressively abandoned nuclear energy.
1975-1980: A Period of Uncertainties
The number of construction projects started for new reactors reached its peak in 1976, with 44 new reactors. But, at the same time, several US utilities began cancelling orders and after 1978, there were no new reactor orders in the country until the four AP1000 units at V.C. Summer and Vogtle in 2013.
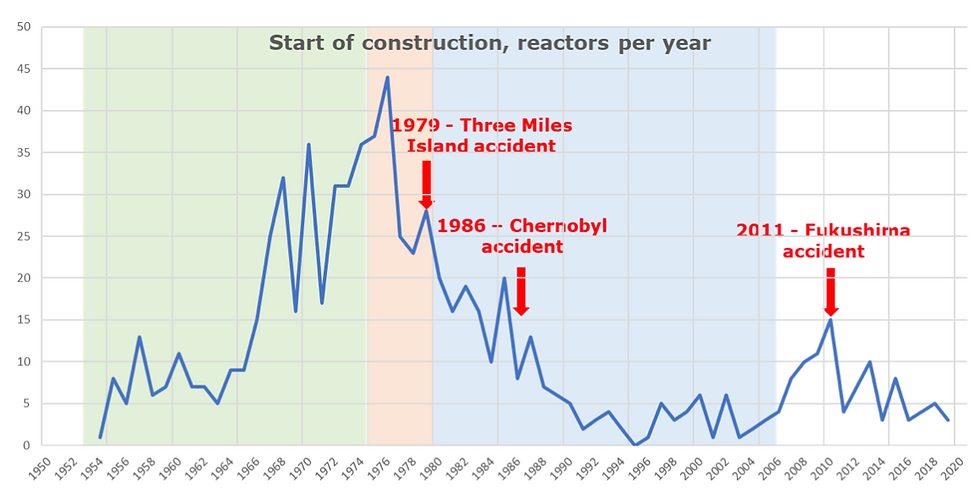
Figure 4: Data IAEA - PRIS
Several factors explain the move of American utilities away from nuclear energy:
The significant improvement in coal and gas plant performance for electricity generation, making them, in most areas, more competitive than nuclear.
The burden of the licensing process, which became longer and longer (large delays in getting construction permits and operating licenses), slowing down, in an uncontrolled way, the construction process and immobilizing large amounts of capital without any clear date for payback.
The raising of new questions on the approach to nuclear safety regarding multiple failures and man-machine interfaces, as presented in the 1975 Rasmunsen report, subsequently confirmed by several accidents, some with minor consequences, such as Brown Ferry in 1975, and one with major ones, at Three Miles Island-2, in 1979.
1981 – 2006: Looking for a Way Out
For safety purposes, mandatory in-depth reinforcements of defenses led to additional costs (new systems, core melt mitigation means). To restore the competitiveness of nuclear energy while addressing safety concerns, the top American vendors developed new designs with increased power levels: Westinghouse with the APWR at 1,350 MWe and GE with the ABWR at 1,315 MWe (which later reached 1,700 MWe).
In the meantime, the European vendors did the same: Framatome with the N4 series rated at 1,450 MWe and KWU-Siemens with the Konvoi at 1,475 MWe; both joined their capacities for a further step forward with the EPR, culminating in 1,750 MWe. Similarly, the USSR was putting online a 1,500-MWe LWGR in Ignalina (1983).
At the same time, an opposite trend appeared in the US, supporting the development of smaller reactors. In September 1984, the analyst J. R. Egan published a paper, “Small reactors and the second nuclear era” (Energy – vol. 9), which gave the basis for what are nowadays called SMRs:
“The prefabricated, factory-assembled plants under 500 MWe may alleviate many of the existing socioeconomic constraints on nuclear manufacturing, construction, and operation. In the industrialized world, small reactors could furnish a qualitatively new energy option for utilities. But developing nations hold the largest potential market for small reactors due to the modest size of their electrical systems. These units could double or triple the market potential for nuclear power in this century. Small reactors will both qualitatively and quantitatively change the nature of nuclear technology transfers, offering unique advantages and problems vis-à-vis conventional arrangements.”
In parallel to the APWR development (which was later abandoned), Westinghouse took that way and launched in 1985 the AP-600 project, with DOE support: a “mature, safe, simple, affordable reactor”, with a 600-MWe power output. The approach consisted of simplifying the design by using passive safeguard systems, making use of gravitational forces -after depressurization- instead of pumps for injecting water to cooldown the reactor in case of accident. The process took time to get licensed and, after numerous tests and experiments, it was finally approved by the US-NRC in 1999. With a design now ready for realization, W ran a detailed cost estimate of the product, and discovered it was completely out of the range to have any chance of entering the market in competition against coal and gas plants.
To get out of the trap, W reshuffled the reactor in less than two years, using the classic trick for making a nuclear reactor competitive: ramp up the power level! And the AP-1000 was designed with a power increase of 85%, while the costs only grew by 12%. Most of the technical solutions of the AP-600 were kept (passive systems, modularization) for shortening the licensing period, and costs were kept under control by growing the reactor, mostly in height, and drastically reducing the margins. The AP-1000 got its final design approval in 2004 from the US-NRC.
2007- Nowadays: A Qualified Renaissance
With growing concerns about global climate warming, it appeared that nuclear energy was one obvious answer for decarbonizing electricity generation while keeping the capacity to produce electricity on demand. This gave momentum to the construction of new reactors.
The Fukushima accident in 2011 broke the new dynamics, as shown in Figure 4; the curve also reflects the up-and-down post-Fukushima uncertainties, with China reassessing its nuclear program and several “newcomer” countries starting construction (Turkey, Bangladesh, Egypt etc.).
Besides that, the difficulties in completing the construction of several reactors, EPR and AP-1000, in Europe and in the USA did not help to strengthen already wavering investor confidence.
Since 2007, 87 reactors have been put into construction, China having the lion’s share with 42 reactors. Most of them are above 1000 MWe, and only 4 of them are in the SMR range: the Chinese Shidao Bay HTGR (200 MWe), the Argentinian CAREM-25 PWR (25 MWe), and the two Russian floating reactors (PWR, 32-MWe), keeping the predominance of large reactors, despite the growing interest in SMRs.
As a Matter of Conclusion, in Expectation of the Next Episode...
To conclude this first part on SMR development, it is important to underline that this is an old idea, which up to now has had limited success due to a huge economic cliff to be overcome. As an example, the US DOE has continuously funded conceptual designs of small reactors since 1955, without any clear success story, each time with the same process: when the industry takes the project over on its own, the project fails due to a lack of funding of the subsequent steps.
It comes from, on the one hand, the amount of investment and the time to the first realization, and on the other hand, the height of the cliff. If applying a usual industrial scaling factor for downsizing reactors from 1,500 MWe to 150 MWe, the cost per kW is multiplied by a factor of about 4. So, in order to be competitive against other electricity sources or large reactors, the scaling factor must be compensated by innovations and breakthrough features. To achieve this, SMR promoters are currently proposing various types of solutions:
Shifting the paradigm (new concept) or simplifying drastically the safety and ancillary systems and benefiting from inherent safety characteristics.
Developing modularization and in-factory realization.
Taking advantage of serial constructions.
Targeting short construction times.
Reducing front-end and siting costs.
Requesting a lightened licensing process and safety framework stability.
Among the 55 designs recorded by IAEA, there is large variety in their development status, and, consequently, there are large uncertainties in assessing their final cost. Some of them use proven technologies, others completely new ones, requesting long and uncertain periods of tests and experiments. All of them need sustainable funding before commercial deployment. In the next letter, we shall scrutinize the proposed designs and analyze the pros and cons that may lead to success.
Thanks for a very interesting article and description of nuclear reactors evolution! Its focus on relations btwn small and big reactors is very valuable in these days. Well, we will see if a new SMR concepts and "breakthrough features" will make them viable more than power sources in remote locations etc.