When ionising radiation ends up saving lives
- Nicolas Mario, Consultant at NucAdvisor
- Nov 8, 2021
- 10 min read
Updated: Dec 8, 2021

Nuclear physics developments during the last century contributed to major technological advances in the energy, industry and the health sectors. This series of papers provides an overview of the healthcare applications of ionising radiation, from its historical developments to its future challenges and opportunities.
In this Part 1, we introduce the different uses of ionising radiation in medicine: both for diagnostic and therapeutic applications. We present an overview of each type of radiation used in medicine, looking at its historical development from discovery to the most recent applications, while highlighting the specificities and challenges, which will be discussed in more detail in future parts.
Part 1 - One century of technological innovations to serve medicine and public health
The revolutionary prospects of X-ray in human body imaging
Until the introduction of X-rays in medicine, physicians had no means to visualize the inside of a patient’s body, drastically limiting diagnostic capabilities. The revolutionary innovation of medical imaging began with the invention of radiology, based on Wilhelm Röntgen’s discovery of X-rays in 1895.
Between the first X-ray pictures taken by Röntgen (see Figure 1 below) and the modern X-ray radiography systems, the imaging principle remained mostly unchanged. An X-ray beam is passed through the patient’s body, some of the X-rays are absorbed or scattered by the internal structures (organs, bones, etc.), while the remaining ones are transmitted to a detector. Radiology can simply be seen as a “shadow picture” technique, using matter density variations within the body to create a picture: the denser the tissue, the more the X-rays are attenuated.
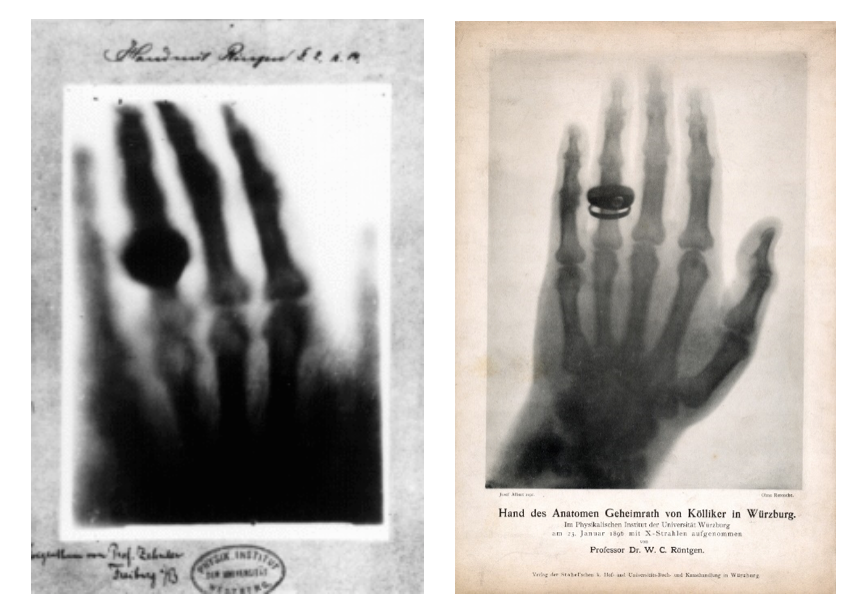
Figure 1: (Left) Hand mit Ringen (Hand with Rings): print of Wilhelm Röntgen’s first “medical” X-ray, of his wife’s hand, taken on 22 December 1895 and presented to Ludwig Zehnder of the Physik Institut, University of Freiburg, on 1 January 1896
(Right) Print of A. von Kölliker’s hand radiography, taken during the lecture Wilhelm Röntgen gave on 23rd January 1896 to the Physical Medical Society of Würzburg.
Within a few years, X-ray imaging established itself as a mandatory procedure in medicine. Different evolutions of the initial technology contributed to expand its use:
the introduction of contrast medium (i.e. use of elements to improve X-rays absorption and enhance contrast) allowing to identify organs with similar X-ray absorption properties;
the development of dedicated X-ray imaging equipment (e.g. mammography systems for breast imaging);
the development of continuous X-ray imaging (Fluoroscopy), allowing real-time imaging during interventional procedures or to visualize specific organs through the propagation of a contrast agent (i.e. blood vessels in angiography).
From planar to 3D imaging: the digital revolution
After decades of technical improvements, the next revolution of X-ray imaging came with computer expansion in the 70’s, leading to modern medical imaging.
Digital X-ray imaging derived from traditional analogic X-ray imaging through the replacement of detection film with a detector sensor (semiconductor, scintillator) linked to a computer able to directly generate of picture. From this point, X-ray imaging became capable of rapidly acquiring multiple images, paving the way for 3D imaging.
The principle of computer tomography (CT) was outlined only a few years after Röntgen’s discovery: The X-ray source and the detector could move around the patient’s body, allowing the acquire multiple images to be ultimately compiled into a single cross-sectional image. A translation of the Gantry system (source + detector) along the patient would then allow to acquire a series of cross-sectional images to be merged into a 3D image of the patient (see Figure 2).
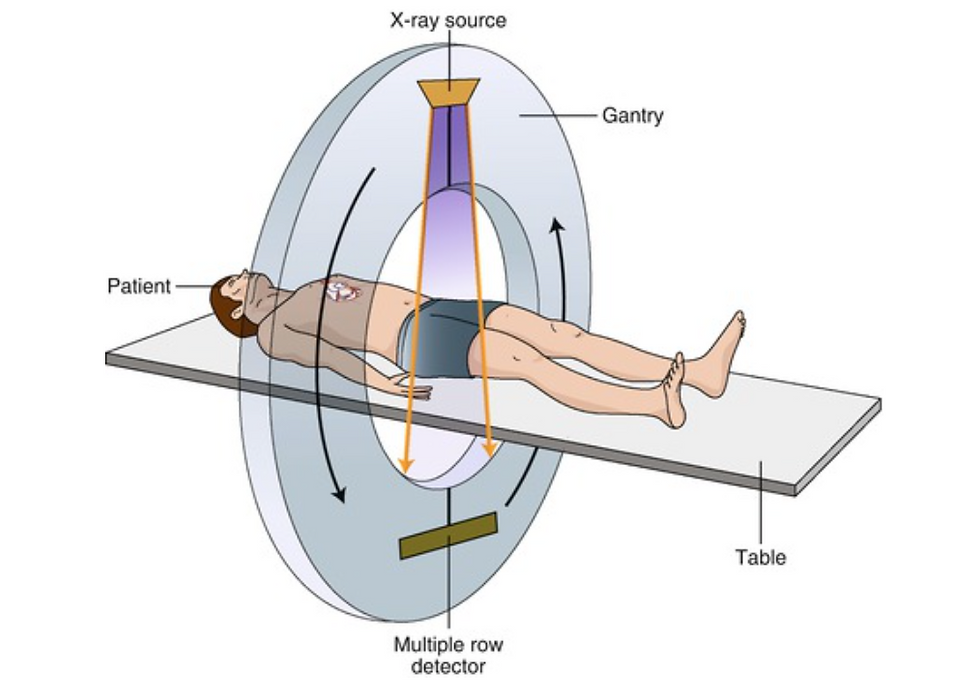
Figure 2: Principle of CT scanner: the gantry is rotating around the patients along with moving all the way through the patient while acquiring multiples images of the body
The impact of planar and CT X-ray imaging in healthcare cannot be overestimated. Despite being more complex and costly than planar imaging, CT scanning quickly became an essential tool for non-invasive examinations, surgical or treatment planning, etc.
Risks associated with X-rays exposure are well-known and addressed by physicians, equipment manufacturers and health/radiation protection regulators. The principal challenge in modern X-ray medical imaging remains the improvement of dose monitoring and the continuous efforts towards decreasing the dose received by the patient.
Nuclear medicine: the birth of functional imaging
Scientific advances progressively provided physicians with a wide range of diagnostic techniques, using ionising radiations (CT or Planar radiography) or others physical properties (Ultrasounds in the 60’s, Magnetic Resonance Imaging in the 70’s) to produce detailed images of internal structures of the body (organs, bones, etc.). However, following progress in Medical Physiology, physicians had to supplement their “structural” diagnostic techniques with “functional” ones, able to assess biological functions and mechanisms.
Radioactive tracers were early seen as a promising tool to monitor bodily functions, with first studies with 210Pb and 210Bi on animals conducted in 1924. Scientific progress in nuclear physics, biochemistry and external instrumentation finally led to the birth of “functional” imaging in the 60’s-70’s with two main techniques used in diagnostic nuclear medicine: Single Photon Emission Computed Tomography (SPECT) and Positron Emission Tomography (PET).
Both techniques are based on the same principle:
A radionuclide is selected for its decay properties (γ-emitter for SPECT imaging and β+ for PET imaging), its chemical properties (binding specificities, toxicity, etc.) and its convenience of use (half-life, supply chains, production cost etc).
The radionuclide is attached to a carrier molecule, such as a protein or a peptide (called vector) that has natural binding properties with the organ or function of interest. The radiopharmaceutical (see figure 3 for detailed view on its principle) is then introduced in the patient’s body (injection or inhalation). After a certain period of time, the radiopharmaceutical has started to accumulate itself in an organ or cell-type of interest and the diagnostic imaging can start. This will either allow to locate specific cells within the body (e.g. cancerous cells in the case of metastatic cancer in the whole body) or to follow a specific bodily function (e.g. blood flow).
An external imaging equipment is then used to monitor the radionuclide decays. For SPECT examinations, a gamma-camera is counting the photons emitted during the decay while performing rotations around the patient. For PET, principle is slightly different, the β+ decay ultimately leads to the emission of two photons that are counted by two detectors in opposite directions continuously rotating around the patients. In both cases the result is a 3D distribution of points indicating the position of the radiopharmaceuticals.
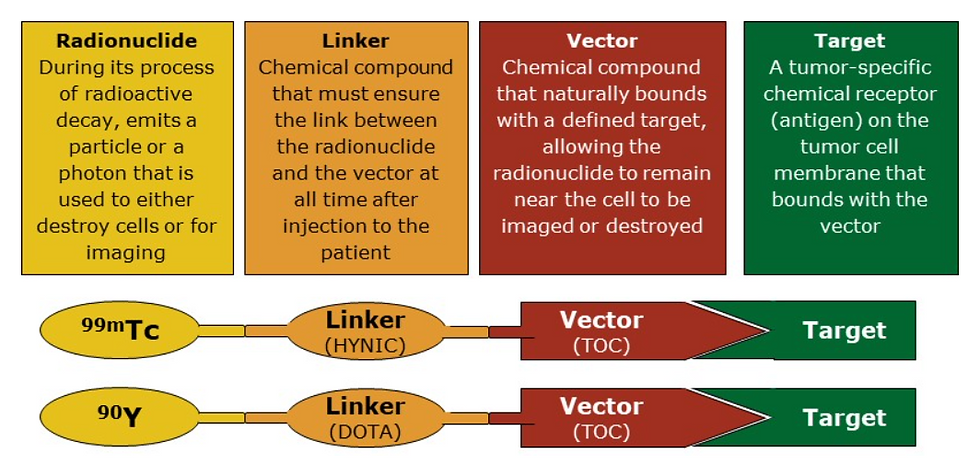
Figure 3: Radiopharmaceutical principle
Nuclear medicine has progressed through the discovery of new radionuclide/vector combinations. Radiopharmaceuticals based on 99mTc and 18F are the widest used ones, respectively in SPECT and PET imaging that represent globally more than 50 million procedures per year across several fields (cardiology, oncology, neurology, etc), to be compared to billions of radiology procedures performed per year.
As compared to other imaging techniques (X-Ray, MRI, Ultrasounds) that rely on a single equipment to be installed and operated over long periods, nuclear medicine necessitates a continuous supply of radionuclides. There are several types of supply chains, either from onsite decentralized production (e.g. in a cyclotron inside or close the hospital), or through international centralized production means for long half-life radionuclides (e.g. research reactors). Each of these supply chains models raises specific challenges, in terms of stable isotopes supply for targets, irradiation means availability, transportation, etc.
The specificities of radionuclides supply chains will be discussed in the next paper.
Complementarity exists among diagnostic technologies
An important complementarity nevertheless exists between CT and SPECT or PET. As stated, diagnostic nuclear medicine enables to follow biological processes at organs or cells scale, and map a certain activity (blood flow, sugar consumption, etc), but SPECT/PET functional imaging output doesn’t provide images of the organs, making it impossible for physicians to precisely locate for example a tumour location. In most cases SPECT/PET examinations are then combined with CT, and results are merged (see example in Figure below).
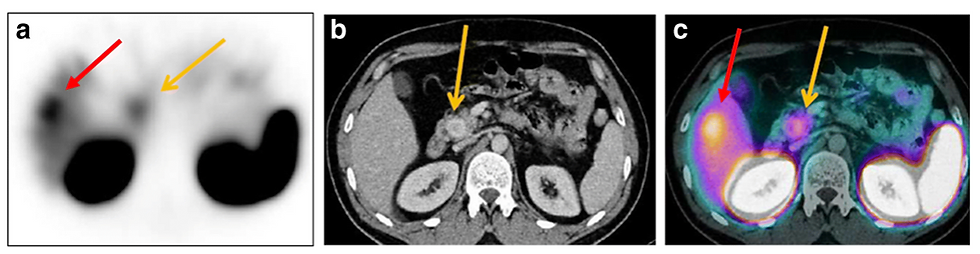
Figure 4: Example of dual SPECT/CT use for neuroendocrine tumour identification.
(a) A scintigraphy SPECT shows two foci of intense tracer uptake in the upper abdomen, (b) localized by contrast-enhanced CT to a hypervascular primary lesion in the head of the pancreas and an isodense metastasis in the right lobe of the liver (arrows), (c) confirmed on SPECT/CT) – Source [3]
Nowadays, with the introduction of dual imaging equipment (PET/CT, SPECT/CT, PET/MRI, etc.), examinations are even performed in parallel, saving time for physicians while improving overall diagnostic capacity.
The frequency at which all these diagnostic technologies are used varies among countries and remains closely linked with professional organisation’s recommendations and guidelines, national regulations and standardized medical protocols, national reimbursement mechanisms, but most importantly with the type of diagnosis pursued by physicians.
All these non-invasive diagnostic techniques are complementary, and their benefits/risks balance are closely monitored by health authorities. For instance, the ionising radiation applications inevitably expose patients to a wide range of doses (from a few μSv for a dental X-ray and 5-10 mSv for a CT scan according to procedure type) that are extensively followed by radiation protection authorities. The medical sector has continuously been implementing practical measures to reduce the dose impact on the patient, either through improving the protocols, setting dose limits, improving the equipment detection capacity to reduce the dose injected or the patient’s exposition, etc. Overall, the average dose of ionising radiation from medical applications received by the general public remains limited, it represents around 20-40% of the annual total, while the remainder is caused by natural radiation.
Nowadays, exposing patients to relatively small radiation doses allows physicians to identify all sorts of structural and functional health issues.
The therapeutic use of ionising radiations
Almost immediately after the X-rays discovery and its pioneering use in medicine, the deleterious biological effects of high radiation levels were observed by the scientific community. It was then quickly assumed that radiations could be used to destroy cancer cells and the first therapeutic experiments began in 1896. By 1899, the first documented successful skin cancer therapy results were presented by Thor Stenbeck and Tage Sjögren to the Swedish Society of Medicine (see figure below).
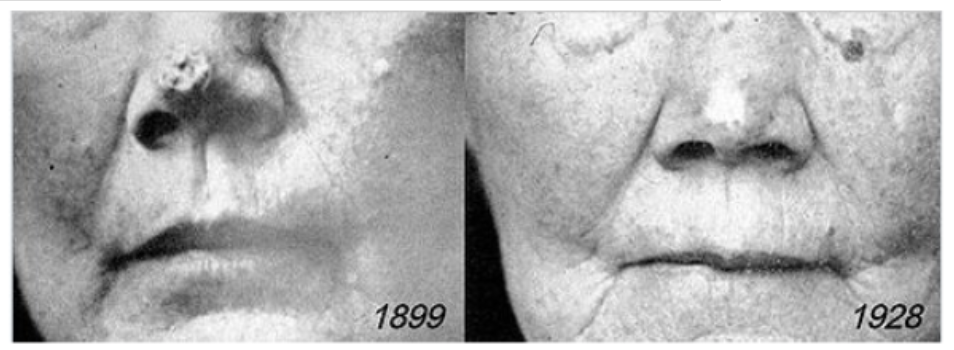
Figure 5: Nose cell carcinoma treated with radiations by Thor Stenbeck, before and 30 years after the treatment
During the 20th century, external beam radiotherapy and brachytherapy (use of a sealed radiation source inside or near the area requiring treatment) progressively developed themselves following advances in medical physics, dosimetry, radiobiology, with some major breakthroughs:
For external beam radiotherapy, the first X-ray tubes (100-500 kV) were only able to treat superficial tumours as the X-rays absorption by human tissue depends on the energy of the beam. Dealing with internal tumours necessitated the development of high-energy X-ray sources, that culminated in the 70’s with the introduction of linear accelerators able to produce high-energy X-ray beams (tens of MeV).
The progressive discovery of a wide range of radionuclides (Cobalt-60, Iridium-192, Iodine-125, etc), that could be easily produced in large quantities in research reactors, led to the replacement of Radium in brachytherapy and contributed to the development of external therapy;
As for the diagnostic applications, the computing revolution allowed physicians to better evaluate and optimize the dose delivered to the patient by modern radiotherapy equipment (stereotactic radiation therapy) through 3D models taking into account type, size and locations of tumours, while improving patients’ safety.
Radiation therapy is now extensively used in cancer treatment, in combination with surgery and chemotherapy. Different beams types are also used or under investigation to offer alternatives to X-Ray, such as protons (proton therapy) or heavy ions (carbon-ions therapy).
Nuclear medicine therapy
In addition to the ionising electromagnetic types of radiation (X-rays and γ-radiation) used in therapy, radionuclides offer additional decay types having potentially even stronger therapeutic efficiency: a stronger destruction to biological systems at a given dose.
Alpha particles have very short ranges in solid materials, (less than 0,1 mm in human tissue) and can be used for small clusters of cancer cells and small tumours.
Beta particles have variable energies and, thus, a distribution of ranges. Given the length of their path through matter, beta emissions can be used for large and/or heterogeneous tumours.
Auger electrons (electrons emitted by radionuclides that decay by electron capture: 111In, 67Ga, 125I, etc.) have lower energy and a much shorter range in material that could allow precise treatments.
The challenge faced by the medical community is to “position” the radionuclides close to the tumours or cancerous cells.
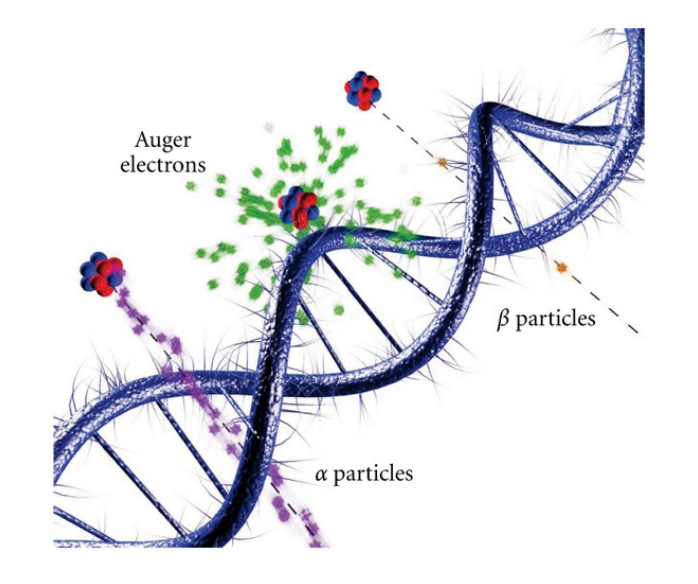
Figure 6: Interactions of ionizing radiations on the scale of DNA – Source [5]
The interest of radionuclides use for targeted therapy was first demonstrated using their natural biological properties. Iodine-131 first treatments against hyperthyroidism occurred in the late 40’s, using the natural ability of the radionuclide to accumulate in the thyroid, and is still widely used to date.
Then, with the development of nuclear medicine, it became clear that radionuclides could also be used to directly target cancer cells and tumours, using the right vector. In targeted radionuclide therapy (TRNT), the radioactive substance bounds directly with specific receptors (target) only present outside or inside cancerous cells (and not only accumulates in a specific tissue/organ), the impact is thus located at the cell level. The main advantage of TRNT is the ability to selectively deliver fatal radiation to cancer cells while causing minimal toxicity to surrounding healthy tissues.
The main difference with conventional therapeutic treatments is the personalised approach towards the patient. As nuclear medicine offers both diagnostic and therapeutic options, it allows physicians to first identify the future efficiency of the treatment through investigating the presence of the specific receptors in cancerous cells (target), with a SPECT or PET procedure, and then only if the target is found, to deliver the radiopharmaceutical treatment. This will have radical impact on health systems, avoiding prescription of several/successive inefficient treatments before finding the one having a positive response.
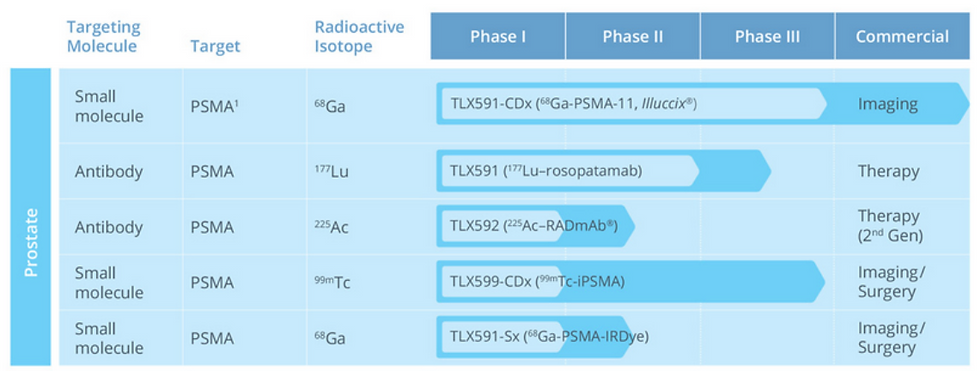
Figure 7: Example of Telix Pharmaceutical pipeline shows that for a single target “PSMA” (Prostate-specific membrane antigen), both treatment and imaging radiopharmaceuticals are in development, this would ultimately allow physicians to directly have diagnostic/therapeutic products pairs
The recent commercial success of radiopharmaceuticals such as Xofigo (based on Ra-223) or Lutathera (based on Lu-177), along with the different company’s acquisitions performed by large pharmaceutical groups in this field in the last decade (AAA, Fusion Pharma, Endocyte, etc.) can be seen as an indisputable sign of the bright commercial future of TRNT.
The overall positive impact of radiation on modern medicine cannot be overestimated
The medical sector largely benefited from advances in nuclear physics during the last century, leading to the development of cutting-edge technologies, supporting diagnostics and treating patients, sometimes through common technologies. Globally, billions of patients are exposed to small doses of ionising radiations every year, with stringent safety and quality standards, while more than one-third of cancer patients are exposed to high doses for treatment or palliative options.
Radiation will undoubtedly continue to play a leading role in the medical sector in the future, considering all the innovations under development, either in terms of equipment, radiopharmaceuticals, etc.
The next paper will specifically address the nuclear medicine sector and its very specific supply chain that is currently at a pivotal time…
Learn more about the different applications of ionising radiation in the medical, industrial and research sectors by reading the following report written by NucAdvisor in the framework of a comprehensive study for the European Commission: “European study on medical, industrial and research applications of nuclear and radiation technology” - https://op.europa.eu/s/sAPx
Bibliography
[1] Berger M, Yang Q, Maier A. X-ray Imaging. 2018 Aug 3. In: Maier A, Steidl S, Christlein V, et al., editors. Medical Imaging Systems: An Introductory Guide [Internet]. Cham (CH): Springer; 2018. Chapter 7. Available from: https://www.ncbi.nlm.nih.gov/books/NBK546155/ doi: 10.1007/978-3-319-96520-8_7
[2] Brian F. Hutton, The origins of SPECT and SPECT/CT, Eur J Nucl Med Mol Imaging (2014) 41 (Suppl 1):S3–S16 - DOI 10.1007/s00259-013-2606-5
[3] Israel, O., Pellet, O., Biassoni, L. et al. Two decades of SPECT/CT – the coming of age of a technology: An updated review of literature evidence. Eur J Nucl Med Mol Imaging 46, 1990–2012 (2019). https://doi.org/10.1007/s00259-019-04404-6
[4] Gianfaldoni S, Gianfaldoni R, Wollina U, Lotti J, Tchernev G, Lotti T. An Overview on Radiotherapy: From Its History to Its Current Applications in Dermatology. Open Access Maced J Med Sci. 2017;5(4):521-525. Published 2017 Jul 18. doi:10.3889/oamjms.2017.122
[5] Lee BQ, Kibédi T, Stuchbery AE, Robertson KA. Atomic radiations in the decay of medical radioisotopes: a physics perspective. Comput Math Methods Med. 2012;2012:651475. doi:10.1155/2012/651475
Comments